Review written by Qiwei Yu (G1, QCB)
Nature never ceases to amaze us, particularly when it comes to how biological organisms develop sophisticated and diverse strategies to survive dynamic and oftentimes hostile environments. Myxobacteria (also known as “slime bacteria”), for example, have evolved a specialized life cycle to cope with the possibility of unreliable nutrient supply. While nutrient abundance enables the bacteria to grow and proliferate, nutrient scarcity can conversely trigger a transition to a more dormant state. In response to nutrient depletion, myxobacteria cells can aggregate into fruiting bodies, three-dimensional multicellular structures of diverse colors and shapes. A subset of the cells within the fruiting bodies develop into rounded myxospores with thick cell walls, waiting for a more favorable environment to resume growth. It is truly amazing how the behavior of a large number of cells can be coordinated to achieve the rapid and dynamic process of fruiting body formation.
Before generating fruiting bodies, which are three-dimensional structures, the myxobacteria cells live in a two-dimensional monolayer. This single layer of cells coats the underlying substrate, the material providing nutrients on which the cells grow. Sequential formation of additional cell layers is the first step in the transition from the two-dimensional monolayers to the three-dimensional fruiting bodies. To understand this process, Princeton researchers Drs. Katherine Copenhagen and Ricard Alert, led by Profs. Ned Wingreen and Josh Shaevitz, have recently combined experimental and theoretical approaches to elucidate the mechanism that controls layer formation in the model organism Myxococcus xanthus (a species of myxobacteria).
Fruiting body formation is a striking example of how a large number of cells can spontaneously organize into complex structures. This process has been of interest to microbiologists for a long time, but its rapidity has prevented detailed interrogation. Here, Copenhagen et al. were able to circumvent this difficulty by controlling the bacterial colony such that it could form new cell layers and holes, but not fruiting bodies (a technique enabled by previous research from the Shaevitz group and collaborators). They then imaged the colony using a three-dimensional laser scanning confocal microscope with subcellular resolution. The imaging revealed that the colony formed discrete layers, and the rod-shaped bacterial cells are densely packed and aligned with neighboring cells. Incredibly, they form a new state of matter called an active nematic liquid crystal, which exhibits rich collective behaviors. The cells are aligned, except at singular points known as topological defects (red and blue symbols in Figure 1). Depending on how cells are arranged around them, the defects can be classified into two categories, which are distinguished by a quantity known as the “topological charge”. In the bacterial colony, the researchers found defects with charge of either +1/2 or -1/2. Moreover, new layers usually form at +1/2 defects, while new holes form at -1/2 defects. In other words, topological features of the bacterial colony seem to determine the formation of new layers and holes.
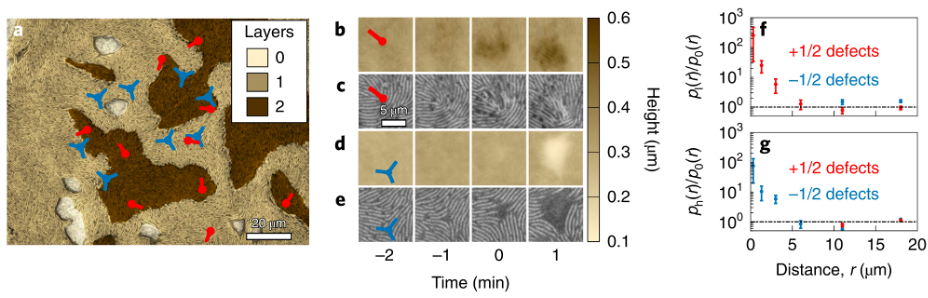
To turn their experimental observations into quantitative understanding, the authors constructed a theoretical model that treats the colony as an active nematic liquid crystal. This model incorporates the active movement of cells. More specifically, the model includes active stresses due to cell motility and mechanical cell-cell interactions in an attempt to predict how this living fluid behaves. In line with their experimental observations, the model predicts cells flowing towards the +1/2 defects to create additional layers and away from the -1/2 defects to create holes. More quantitatively, the theoretical model also provides predictions of the cell velocity near the defects, which enables the researchers to predict how cells move before generating holes or new layers. To test the velocity predictions produced by their model, Copenhagen et al. measured the actual velocity profiles in their experiments. Excitingly, the measurements were in agreement with the predictions of the model. This suggests that the theoretical model paints a consummate picture of how cell motility and mechanical interactions drive the formation of multilayered structures, which is the precursor to fruiting bodies.
It is particularly noteworthy how topological defects get involved in complex biological processes such as myxobacteria fruiting body formation. To many, topology sounds like an advanced and obscure mathematical theory that bears little relevance in real living and physical systems. For physicists, however, topology is hardly a new concept. In fact, topological phases of matter have long been a hot topic in condensed matter physics. The groundbreaking work in this field, the Kosterlitz-Thouless transition, involves the association and dissociation of positive and negative defects very similar to the ones observed in myxobacteria colonies. Indeed, these mathematical and physical concepts are becoming increasingly useful in understanding many complex types of biological matter. The abstraction of complex biological processes into simple physical principles enables us to understand, manipulate, and engineer living systems in a more rational and systematic fashion.
This comprehensive study combined biological experiments, computational analysis, and physical modeling to achieve a comprehensive understanding of myxobacteria layering. In addition to helping us better understand the behavior of myxobacteria, it also demonstrates how combining interdisciplinary expertise could be extremely useful in the investigation of complex systems, such as life.
The lead authors Drs. Katherine Copenhagen and Ricard Alert remark upon this topic when asked about what was critical to the success of their study. They emphasized that the culture of close collaboration between experiment and theory at Princeton was a major driving force in this project, especially through the Center for the Physics of Biological Function (CPBF). In addition, the Keyence VK-X 1000 microscope, which was at the time at the Princeton Institute for the Science and Technology of Materials (PRISM), was an integral tool to their discoveries. They are now actively exploring the continuation of this project in several directions, such as how multiple layers form and stack up to eventually form the fruiting bodies, how to understand the traction forces that cells exert on the underlying substrate as they move around and form new cell layers, and how to tune the activity of the bacteria to experimentally observe active Kosterlitz-Thouless transition in the lab.
The original article was published in Nature Physics on November 23, 2020. Please follow this link to view the full version.