“Rediscovery” of a decades-old physics idea reignites the fields of cellular and molecular biology
Review written by Xinyang (David) Bing (LSI)
Lava lamps are fluorescent mixtures of oil and water that are immiscible and, when heated, float around, generating hypnotizing patterns that lull you to sleep. Now, biologists are seriously considering the possibility that the same physics that govern lava lamps may also control almost everything that goes on inside our cells.1 Walk through the halls of MIT and Harvard, Oxford and Cambridge, or of course Princeton, and you would likely hear what everybody is talking about: liquid-liquid phase separation.
This decades-old concept in the field of physics has now been “rediscovered” in cellular and molecular biology. This rediscovery began with two groundbreaking publications, first by Cliff Brangwynne followed by Steve McKnight, who described the biophysical properties of protein complexes that separate out from solution and form gel-like “condensates” (Fig. 1). They proposed that the basic physics underlying this liquid-liquid phase separation, or simply, phase separation, could control most every other aspect of cell biology.2,3
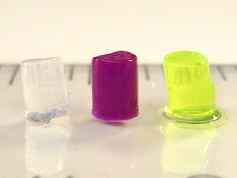
About a decade later, Cliff’s and Steve’s predictions have been realized. Phase separation has been implicated in controlling a number of biological processes, including the timing and intensity of gene transcription, the formation of protein complexes when the cell experiences severe stress, and the production of ribosomes when a cell is growing under ideal conditions.4,5,6 Now, in a recent paper from Princeton’s Brangwynne lab, the potentially tremendous role of phase separation in cell biology has once again been thrust upon the field’s collective intelligence.
In molecular biology, a conundrum has long confounded scientists: the concentrations of proteins that are necessary to study the biochemical properties of enzyme reactions via in vitro protein assays are too high to be physiologically relevant. Perhaps, detractors argue, all findings discovered in vitro are false, or at best represent some artificially magnified version of what actually occurs in vivo. If the detractors are correct, however, our entire understanding of how proteins carry out their functions inside cells would topple upon the weight of these “baseless” measurements.
The critics’ hypothesis fails to fully account for the fact that cells are filled with membraneless organelles, or compartments, that appear to concentrate specific types of protein and RNA complexes. Each compartment or organelle plays a different role in the cell depending on the types of proteins and RNAs they contain. Rather than being aggregates, or a cellular trash can of useless, misfolded proteins and degrading RNAs, these compartments could be what amounts to highly concentrated “enzyme factories”, similar to those found in a tube on a biochemist’s bench. Most importantly, Steve and Cliff postulated that the formation of these “enzyme factories” is self-governed by the physical properties of the proteins and RNAs that make them up: they spontaneously self-associate and condense, or phase separate, into these specific molecular condensates. By this hypothesis, the cell could essentially be considered a lipid bag full of self-assembling, hyper-efficient, “enzyme factories” capable of performing chemical functions at a speed and intensity that is crucial to all life in the universe.
Stress granules and P-bodies
One fascinating example of these condensates is stress granules. Challenged by certain types of acute stress, cells actually form “condensates” or “aggregates” (depending on who you ask), of highly concentrated proteins and RNAs in the cytoplasm. These can be easily visualized under a high-powered confocal microscope as dark spots that appear inside the cytosol. Why cells form these condensates is still an open question, but most researchers in the field believe that these form as a way for cells to concentrate protein assembly machinery, or ribosomes, that have failed to properly generate nascent proteins (also known as stalled ribosomes). Production of new proteins is extremely costly to the cell from an energy and resource expenditure perspective. Therefore, stress granules might be a way for cells to conserve energy under an acutely stressful situation. Essentially, the cells are hoping for a “better day” to come when they can resume their normal cellular activities, i.e., busily creating new proteins...kind of like the COVID19 shutdowns, which David is currently working on to understand better the “mechanistic basis for cell-cell fusion, which is [...] hijacked by viral infections including COVID-19.”
In addition to stress granules, cells also produce another type of condensate associated with stress granules called “P bodies”, which, instead of being storage units for ribosomes, appear to be enzyme factories focused on degrading mRNAs. However, the two types of condensates share protein components, which has led to some controversy in the field about what exactly differentiates P bodies from stress granules, along with how two similar but different enzyme factories can form from overlapping protein components. From the lab of Cliff Brangwynne, post-doc David Sanders set out to understand the rules that govern the formation of these two liquid-like compartments in the stressed cell cytosol.
The nascent field of phase separation, occupied primarily by biophysicists and old school biochemists, largely has been stagnant. Most studies consist of either descriptions of condensate formation using mostly in vitro assays (see Figure 1) and anecdotal evidence, mathematical modeling of these observations using tools with limited range of applicability, or artificial incantations of phase separation using engineered fusion proteins. This has led to fierce criticism from all across the molecular biology landscape, though it centers overwhelmingly on two important points. First, what are the actual in vivo biophysical mechanisms of phase separation? And, secondly, what role does phase separation play in the functionality of these so-called liquid-like condensates? In other words, what drives some protein complexes to form condensates under certain conditions, but not others, and why is this physical property of forming these condensates of protein complexes important for function? Is it really necessary for the function of these protein complexes to be involved in these highly concentrated condensates?
What drives stress-granule and P-body formation?
With a background in psychology, a rather “tortured scientist” demeanor, and a hyper-detail-oriented work ethic, one could say that David Sanders was the right person to answer these challenging questions. Published in Cell, his “highly collaborative” paper, along with two other papers from independent groups published in the same edition, lay out a comprehensive dissection of the biophysical mechanisms underlying the phase separation of stress granules and P-bodies. Specifically, David and his co-authors, including Amy Strom, famous for her work on heterochromatin condensates, and local graduate student Daniel Lee, describe several very surprising and important observations.
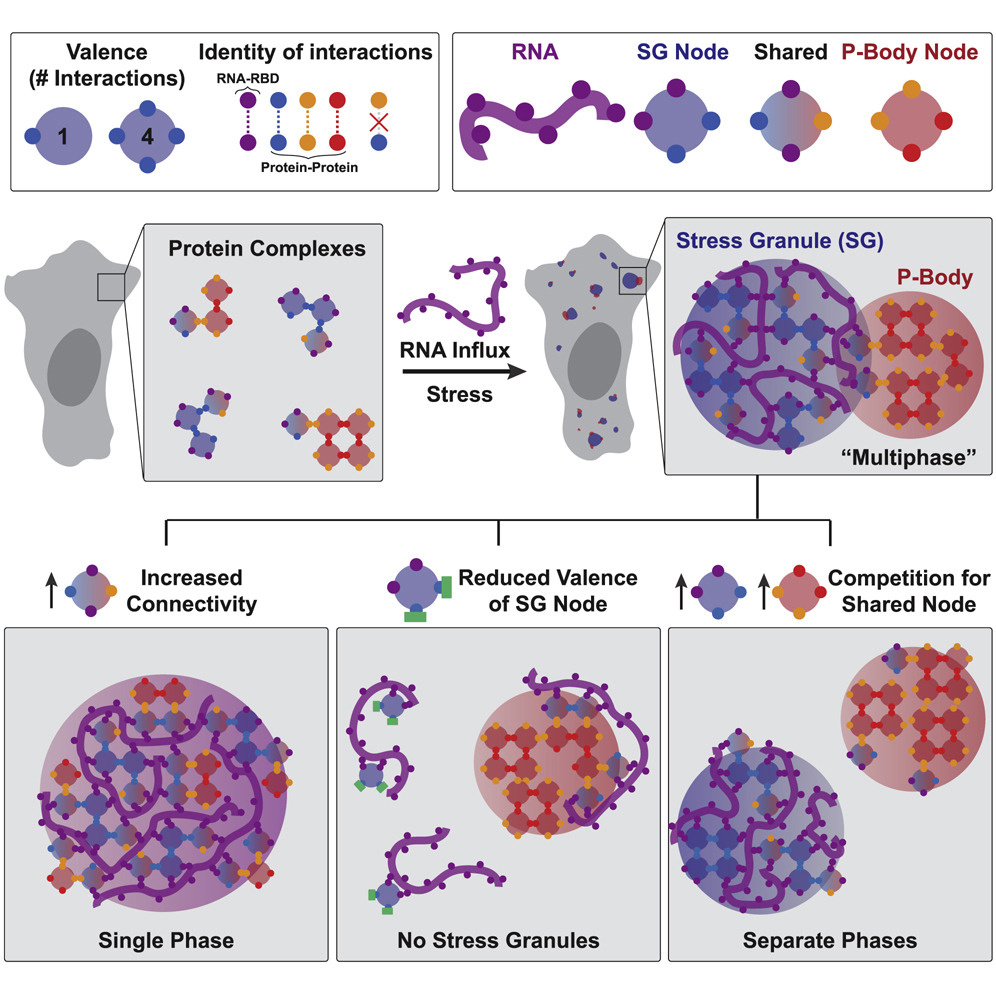
First, they show that the intrinsically disordered regions of proteins that drive stress granules and the formation of P-bodies are not necessary for condensate formation, but rather only serve to control the size of condensates. This is very surprising given that a large portion of the previously published results on phase separation implicate intrinsically disordered regions of proteins as the potential drivers of phase separation. However, because previous studies have largely used in vitro or engineered assays at extremely high protein concentrations to study the biophysical properties of phase separating proteins, their findings might not reflect the actual biological processes underlying phase separation in vivo. In other words, while protein jellies might look cool (Figure 1), they might not reflect what actually occurs in a cell. One must keep in mind, however, that the intrinsically disordered regions studied here reflect only one regime of these types of domains, in one set of protein types, so the generality of the results need to be taken with a grain of salt.
Perhaps the most important contribution by Sanders et al. is the concept that phase separation occurs through network associations (Figure 2). Through comprehensive analysis of various interaction domains of proteins necessary and sufficient for formation of stress granules and P-bodies, David demonstrates that phase separating proteins essentially behave like “nodes” in a network. The domains of the proteins involved in this node behavior can either associate with RNAs or with other proteins in the network (which increases the number of nodes and draws in more of these proteins) leading to the separation of these proteins in the network from the rest of the cytosol, forming a condensate. This behavior is also stoichiometric, meaning that each protein has a finite number of valence depending on the domains it contains. While these concepts may seem intuitive, studies in the cellular phase separation field so far have only hinted at but not demonstrated this type of behavior in liquid-like condensates. This led to an extremely murky understanding of the biophysical mechanisms driving phase separation so far. Importantly, interactions of proteins through their valency in a network lends a powerful and rational explanation of what drives phase separation in cells.
To summarize, the naturally high valency of the proteins necessary for formation of stress granules and P-bodies lead to their preferential binding of the RNAs released from ribosomes during stress. This binding forms condensed ribonucleoprotein networks that appear as phase separated condensates. This explains why RNAs are necessary for phase separation behavior, and also why only some proteins drive phase separation while other proteins don’t even participate in these networks. What’s most important might, in fact, be the number of ribonucleotide binding and protein interaction domains in a protein. This theory also addresses why there is a certain level of biochemical mixing and microscopic association between stress granules and P-bodies; competition between the two networks for shared protein components, or nodes, controls this type of behavior. In addition, protein disorder, which was previously thought to be extremely important for phase separation, might only serve as the cherry on top, not the whole cake. Is all of life really governed essentially by the same physics as lava lamps? You only have to walk over to the Hoyt Building to find out.
The original article was published in Cell on April 16, 2020. The full version may be found here.
References
1Boeynaems et al. Cell 28, 420-435 (2018).
2Brangwynne, C. P. et al. Science 324, 1729-1732 (2009).
3Kato, M. et al. Cell 149, 753-767 (2012).
4Cho, W.-K. et al. Science 361, 412-415 (2018).
5Molliex, A. et al. Cell 163, 123-133 (2015).
6Feric, M. et al. Cell 165, 1686-1697 (2016).